Astronomy Lasers
What are Astronomy Lasers?
Lasers have become an essential tool for astronomers, enabling more precise observations of celestial objects. In particular, they make it possible to create better images of distant stars, galaxies, and other celestial objects than previously possible.
Lasers are used in several distinct areas in astronomy. Most commonly, they are employed in various techniques to improve the imaging quality and capabilities of large astronomical telescopes. But they are also key to gravitational wave sensing, as well as other applications.
Laser guide stars
One major limitation in forming high-resolution images of celestial objects with telescopes is the blurring effects of the Earth's atmosphere. In particular, turbulence and temperature variations in the air column above the telescope distort the wavefront of light coming from astronomical objects, making it impossible to form perfectly sharp images of them.
One way to minimize or avoid this entirely is to place telescopes on very high mountain peaks, or out into space. But the performance of telescopes located on even the tallest terrestrial mountains is still significantly limited by what is called “astronomical seeing.”
One method astronomers have developed to minimize the problem of astronomical seeing is adaptive optics (AO). This uses a flexible or deformable mirror in the telescope optical path to adjust the shape of the wavefront in real-time to correct for the atmospheric distortions.
Adaptive optics utilize the fact that stars are so distant to us that they appear to be point sources producing a perfectly flat wavefront. So, we measure the actual wavefront from a star and calculate how far it departs from being flat. Then, this information is used to tell a deformable mirror how to change shape in order to correct the wavefront and restore it to being flat.
Adaptive optics systems can make thousands of adjustments per second to compensate for the rapidly changing atmospheric distortions. This results in much sharper and more detailed images of celestial objects compared to traditional telescopes.
But AO systems need a fairly bright star in order to work properly. Depending upon where the telescope is pointed in the sky, there may or may not be a sufficiently bright star within the field of view. If there isn’t, then an artificial guide star can be created by shining a laser into the atmosphere. The laser guide star (LGS) can then be used as a reference wavefront for the adaptive optics system.
There are two distinct approaches to actually creating an LGS, and many variations in how each is actually implemented. The most widely used technique utilizes a laser that emits at 589 nm to excite sodium atoms that exist at an altitude of about 90 km in the atmosphere. The sodium atoms absorb and then re-emit the laser light creating the LGS.
The second method relies on what is called a “Rayleigh beacon.” This approach typically uses an ultraviolet laser to produce light scattering from molecules from about 15 – 25 km up in the atmosphere. While Rayleigh beacons are simpler and less costly to construct, they don’t provide a wavefront reference that is as good as the sodium LGS approach. This is because the Rayleigh beacon LGS appears much lower in the atmosphere, and therefore doesn’t experience precisely the same distortion as light coming from astronomical objects.
Multiple telescope interferometry
Another way to improve telescope image quality is to make the aperture bigger. This is because the larger the telescope, the lower the negative effects of light diffraction on image quality. A larger telescope can therefore produce more detailed and brighter images.
But, on the practical side, there are limits to how large we can construct telescopes. One way around this is to combine the light from multiple telescopes to simulate a larger, and therefore higher-resolution, instrument.
To combine their light, the telescopes must be in close physical proximity. Then, the individual beams must be combined with extraordinary accuracy. Specifically, the distance from each telescope to the point of recombination must be the same to within a small fraction of the wavelength of light. For visible light, the wavelength is about 0.5 µm.
But, even if the optical paths for each telescope are nominally identical, real-world effects of thermal expansion and vibration will produce time varying errors in the total path length that are far larger than the required value. To correct for this, “delay lines” are used in the beam path for each telescope. These allow the total path length for each to be minutely and highly accurately adjusted in order in order to keep all the distances exactly the same.
There are a variety of different for schemes for implementing delay lines when combining multiple large telescopes. Frequently, these involve bouncing the beam off mirrors which are mounted on rails. This allows them to be moved along the optical axis. Changing the mirror position adjusts the length of the delay line.
Key to the success of this technique is the ability to measure the position of the mirrors with an accuracy that is a fraction of the wavelength – a few tens of nanometers for visible light. Distance measurement based on laser interferometry provides the ultimate and most sensitive means for accomplishing this. Typically, this uses low power, CW, visible wavelength lasers with a relatively narrow linewidth. This provides the coherence length needed to perform interferometry over a path length of several meters or more.
Other astronomy applications for lasers
There are quite a number of other uses for lasers within astronomy. For example, laser interferometry also forms the forms the basis for gravity wave astronomy.
But, in the case of the Laser Interferometry Gravitational-Wave Observatory (LIGO) – actually two separate observatories located in Hanford, WA, and Livingston, LA – the precision and sensitivity is far beyond anything achieved before.
Each of these facilities utilizes an L-shaped interferometer with arms that are approximately 4 km long. LIGO is sensitive enough to measure a change in path difference between the two interferometer legs to a distance that is less than 1/1000 the diameter of a proton. This is what is necessary to measure the gravitational waves – the small ripples in spacetime – that are produced when black holes collide.
LIGO actually incorporates quite a number of lasers and laser amplifiers. The main beam for the interferometer is produced by a Coherent Mephisto. This laser was chosen because it uses a non-planar ring oscillator (NRPO), which is widely recognized as the lowest noise and narrowest linewidth CW laser architecture. The output of the Mephisto goes through several stages to amplify it, reduce its noise, and stabilize its frequency, power and transverse mode structure.
Lasers are also routinely used to measure the distance from the Earth to the Moon. They do this by bouncing laser pulses off arrays of retroreflecting mirrors left on the lunar surface by three of the Apollo missions, as well as two subsequent Russian lunar rovers. The time of flight or the journey is used to calculate the distance, and precisions of just a few millimeters can be obtained.
Lasers have also made it to Mars on NASA’s Perseverance rover. It uses a laser to vaporize small amounts of Martian rocks. This creates a plasma which emits light. Spectroscopic analysis of this light reveals the chemical composition of the rock.
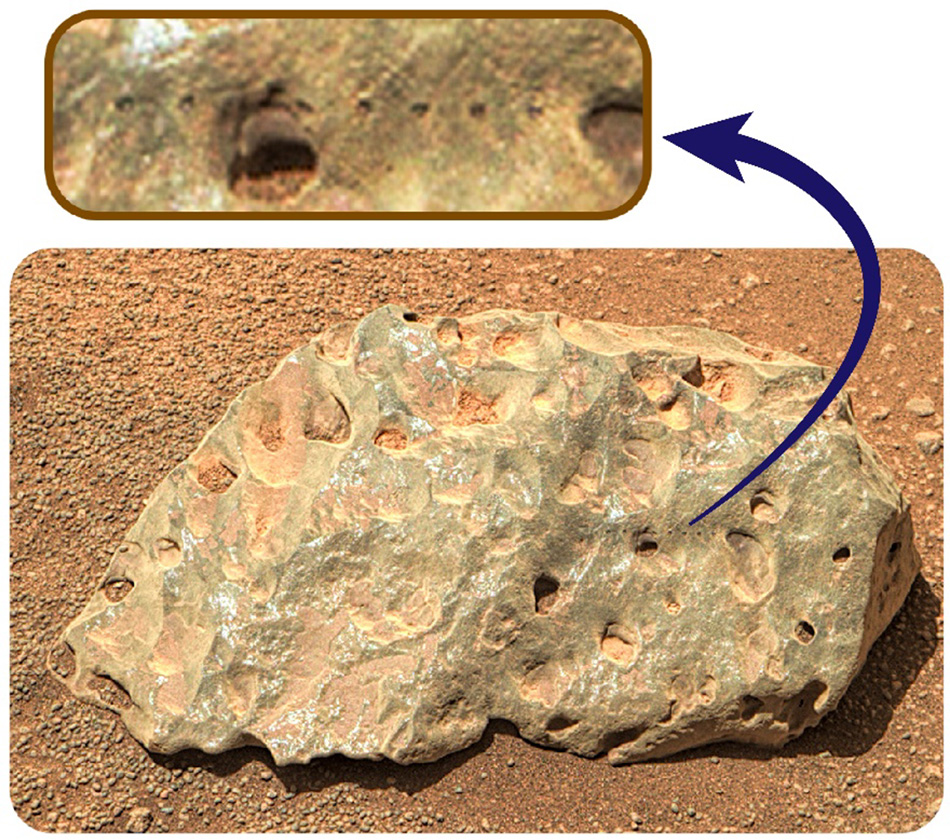
Figure 1. A series of holes created in a Martian rock by the laser on the NASA Mars Perseverance rover. Photo NASA/JPL-Caltech/ASU.
Overall, astronomy lasers play an important role in the advancement of research and observation. They will likely continue to play a key role in the development of new technologies in the years to come.